1.IntroductionTo understand the basic biological functions in a living system one needs a sophisticated detection system. Luminescence technique has been widely used as a sensitive tool for many years in many areas of sciences and technologies.1 The signaling source is a luminescent probe, i.e., a fluorophore. Up to now, most of the fluorophores used to detect biological components in the living systems are organic dyes. To a large extent, the use of these dye molecules has greatly helped the understanding of many biological problems. However, the photobleaching of these dye molecules has been a severe problem. Sensitive detection and real-time monitoring of important biological processes require continuous exposure of an intense excitation source under which the use of organic fluorophores is unsuitable. Therefore, there is a demand to develop nonisotopic, highly photostable and sensitive luminescent probes.2 3 4 5 6 7 Recently, the use of semiconductor quantum dots has shown great promises due to their unique properties, e.g., size-tunable luminescent properties and functionalizability to couple biomolecules.2 3 7 The development of such a new generation of biomarkers has been suggested to be better than organic dyes. Nonetheless, they have not been used extensively because of their poor solubility in water, agglutination, blinking properties and moderate quantum yields. Fluorescent latex particles such as polystyrene particles have also been used in some biological applications.8 9 10 11 However, they are not very suitable for ultrasensitive biochemical analysis because of the following limitations: agglomeration, swelling, and dye leaking. The development of nanoparticle based luminescent markers has become a challenging field of research in recent years as such types of nanoparticles can be engineered to obtain desired luminescent properties. In an effort to synthesize highly stable, sensitive and uniform biomarkers, we have developed novel dye doped silica (DDS) nanoparticles for application as an efficient biomarker (Figure 1). The incorporation of a relatively stable inorganic dye, Rubpy, into the silica network prevents dye photobleaching, communication to the outside environment and dye leakage. The silica matrix not only protects the dye but also provides some unique features, which are very important while working with biological samples. For example, silica is a biocompatible substance and extremely stable in an adverse environment. Moreover, silica nanoparticles form a clear dispersion in water and their surface can be modified easily to attach biomolecules through many existing molecular immobilization mechanisms.12 13 14 Our DDS nanoparticles are uniform because they are made from water-in-oil (W/O) microemulsion, a medium that controls the size of the particle. To demonstrate the labeling efficiency, we have modified the DDS nanoparticle’s surface biochemically to attach membrane anchoring lauroyl groups and used them for human leukemia cell staining. 2.Experiment2.1.MaterialsTris(2,2′-bipyridyl)dichlororuthenium II) hexahydrate (Rubpy), tetraethylorthosilicate (TEOS), trimethoxysilylpropyldiethylenetriamine (TSPDT), Triton X-100 (TX-100), lauroyl chloride, fluorescamine, n-hexanol, tetrahydrofuran, dimethylsulfoxide, and cyclohexane were purchased from Aldrich Chemical Co. Inc. Ammonium hydroxide (28–30 wt) was obtained from the Fisher Scientific Co. All other chemicals were of analytical reagent grade. De-ionized (DI) water (Easy pure PF, Barnstead Co.) was used for the preparation of all solutions. 2.2.Synthesis of DDS NanoparticlesW/O microemulsion was prepared first by mixing TX-100, cyclohexane, n-hexanol (1:4.2:1 V/V) and water. Figure 1(A) shows a schematic representation of W/O microemulsion system (co-surfactant molecules were not shown) where the water pool serves as a nano-reactor. The role of n-hexanol was as a co-surfactant to the nonionic surfactant, TX-100. The molar ratio of water to surfactant was defined as W0 value. Two different W0 values, 5 and 10, were chosen for the synthesis of small and medium size particles, respectively. One hundred microliters of distilled tetraethylorthosilicate (TEOS) was added into the 10 mL microemulsion solution and polymerization reaction was then initiated by adding ammonium hydroxide (NH 4 OH). The volume ratio of pure TEOS to NH 4 OH (28–30 wt) was 1.7. The dye stock solution was prepared in DI water. The overall dye concentration in microemulsion was varied from 0.0 M (pure silica nanoparticles) to 1.2 mM (maximum dye loaded DDS nanoparticles). The silica polymerization reaction was allowed to continue for 24 h. This polymerization reaction scheme is shown in Figure 1(B). For the synthesis of large size DDS nanoparticles 100 μL of TEOS was added further in the microemulsion (W0=10) and the particle growth process was allowed to continue for an additional 24 h. Both pure and dye doped silica nanoparticles were then isolated from the microemulsion by adding acetone, centrifuging, washing by ethanol, water for several times (in order to completely remove surfactant molecules) and finally drying over acetone. Postsilica coated nanoparticles were also made by dispersing dried DDS nanoparticles first in microemulsion (W0=10) followed by the addition of the required amount of TEOS and NH 4 OH. Both the amount of TEOS and the polymerization reaction time control the thickness and thus the overall size of the postsilica coated nanoparticles. The thickness of the postsilica coating can be varied from 2 to 170 nm. 2.3.Surface Modification to DDS NanoparticlesThe silica surface of DDS nanoparticles was silanized by trimethoxysilylpropyldiethylenetriamine (TSPDT). A freshly prepared 1 (v/v) solution of distilled TSPDT in 1 mM acetic acid was treated with the medium size DDS nanoparticles (20 mg/ml) for 30 min at room temperature (23 °C). The TSPDT modified particles were then thoroughly rinsed with de-ionized water and centrifuged to remove excess TSPDT. The availability of the free primary amine groups on the silica surface was confirmed by the fluorescamine assay (see Section 3.1).15 The amine-functionalized particles are then treated with lauroyl chloride in dry tetrahydrofuran in the presence of argon atmosphere for 6 h.16 These particles showed negative fluorescamine assay indicating that amine groups were consumed. Figure 1(C) shows the schematic representation of surface modification to DDS nanoparticles. 2.4.Membrane Probing ExperimentFive milligrams of medium size lauroyl derivatized DDS nanoparticles were dispersed ultrasonically in 10 ml DMSO and treated as stock solution. Human leukemia cells (mononuclear lymphoid cells, about 2 millions/ml) were obtained17 as a suspension in the cell medium and 100 μL of this suspension was diluted with 2 mL phosphate buffer (PBS) (pH 6.8). Forty microliters of lauroyl derivatized DDS nanoparticles in DMSO were added to the cell medium and incubated for 6 h. Final concentration of DMSO in cell medium was 2 (V/V). No change in cellular morphology was observed with this DMSO concentration. After incubation, we observed that leukemia cells were sedimented at the bottom of the vial. We decanted out the supernatant very carefully and then added PBS buffer. Cells were thoroughly washed with the PBS buffer to completely remove unbound DDS nanoparticles using both vortex and centrifugation techniques. One has to be very careful during the centrifugation step to avoid cell damage. After thorough washing both optical and fluorescence images were taken by a fluorescence microscope. 2.5.InstrumentationLuminescence emission spectra both in solution and solid state were recorded on SPEX Fluorolog-τ2 Spectrofluorometer (Instruments S.A. Inc.) operated in the steady-state mode. The size of the nanoparticles was measured by the Hitachi transmission electron microscope (TEM, model H7200). Optical and fluorescence images were taken by an inverted Olympus fluorescence microscope (model IX708F) assembled with an intensified charge coupled device (CCD) camera. Eppendorf centrifuge (model 5810R) was used for high-speed centrifugation. 3.Results3.1.Nanoparticle Characterization3.1.1.TEM MeasurementTEM images show an excellent uniformity of DDS nanoparticles, 5±1 nm for small (I), 63±4 nm for medium (IIa) and 400±10 (III) for large size particles (Figure 2). The analysis of medium size DDS nanoparticles at high resolution TEM (IIb, Figure 2) shows dye molecules as black dots embedded inside the silica network. This is due to the presence of heavy metal atom in the dye. Pure silica nanoparticles do not show such black dots at the same TEM resolution. 3.1.2.Spectroscopic MeasurementThe emission spectra of the pure Rubpy and medium size DDS nanoparticles were measured in both aqueous solution and in solid state. Pure Rubpy showed emission at 594 nm when excited at the 450 nm in aqueous solution. In the solid state the emission spectra were different from the solution phase (Figure 3). The emission band maximum of the pure Rubpy appeared at 576 nm [Figure 3(b)]. In case of DDS nanoparticles, the complete spectrum shifted towards the longer wavelength and the emission band appeared at 608 nm [Figure 3(c)]. The emission spectra for the postsilica coated DDS nanoparticles were slightly redshifted and the band maxima appeared at 610 nm [Figure 3(d)]. Emission spectra of both DDS nanoparticles and postsilica coated DDS nanoparticles in aqueous suspension were similar to those recorded in the solid state. 3.1.3.Photobleaching ExperimentRubpy is also known to be a photosensitive compound. To investigate whether the Rubpy molecules still remain photosensitive when doped inside the silica network, we have recorded emission spectra in the solid state with respect to time (Figure 3). Four different samples were selected for this experiment: Rh6G [Figure 3(a)], pure Rubpy [Figure 3(b)], medium size DDS nanoparticles [Figure 3(c)] and postsilica coated DDS nanoparticles [Figure 3(d), thickness of the silica coating was about 10 nm]. A 450W Xenon lamp was used as an intense excitation source, and each sample was continuously exposed at maximum excitation band pass (14.7 nm). Emission spectra were recorded at every 5 min interval for a period of 1 h. Figure 3(a) shows that Rh6G fluorescence intensity decreases very sharply. When calculated using the data obtained at 5 min and at 1 h time, the total decrease in intensity was 56.4 and 80.8, respectively. As an inorganic dye, Rubpy is more photostable than Rh6G. Its photostability further increases when doped inside the silica matrix. As expected, 55.9 and 31.8 decreases in the initial intensities were observed for pure Rubpy and DDS nanoparticles after a 1 h period of continuous exposure, respectively [Figures 3(b) and 3(c)]. We realized that even in DDS nanoparticles, dye molecules were not completely protected from the outside oxygen penetration and hence photobleaching. We have thus used a postcoating procedure to add a thin layer of silica onto the DDS nanoparticle surface. The idea of postsilica coating has twofold advantages: (i) it can completely protect dye molecules from photobleaching, (ii) it will provide 100 pure silica surface for efficient surface modification. Our results, shown in Figure 3(d), clearly demonstrate that the postsilica coated DDS nanoparticles were highly photostable when compared to other samples. The initial luminescence intensity was decreased only by 5.6 after 1-h excitation. This observation, therefore, proves that additional silica coating completely isolates dye molecules from the outside environment and therefore completely protects the dye molecules. 3.1.4.The Effect of Increase of Dye Concentration on the Luminescence Intensity of Medium Size DDS NanoparticlesTo investigate how much dye can be loaded inside DDS nanoparticles to achieve maximum luminescence intensity, we have monitored luminescence intensity with respect to the increase of dye concentration. Medium size DDS nanoparticles were chosen for this study. We have synthesized DDS nanoparticles first by varying the dye concentration from 0.04 to 1.2 mM with respect to the total volume of microemulsion. Luminescence intensity was then measured at a particular concentration (0.01 mg/mL, ultrasonically dispersed) for each sample. We observed that dye-loaded nanoparticles with about 20 wt (corresponds to ∼0.7 mM dye concentration; calculated by weight measurement taking pure silica nanoparticles as reference) had the highest emission intensity. Further dye loading reduced luminescence intensity due to self-quenching of dye molecules. We have also studied the oxygen effect on the luminescence of the nanoparticles. Pure Rubpy shows significant luminescence quenching with the increase of oxygen concentration and therefore is used as an oxygen sensing reagent.18 We have not observed any quenching of DDS nanoparticle luminescence up to 8 psi air pressure. The luminescence intensity was decreased only when a higher air pressure was used. 3.1.5.Distribution of DDS Nanoparticles in PMMA Thin FilmHigh resolution TEM image (IIb, Figure 2) of medium size DDS nanoparticles showed that dye molecules are distributed throughout the entire silica network. Each black dot could be the contribution of more than one Rubpy molecule. We were able to see the contrast because of the presence of heavy metal ruthenium. The dimension of each black dot is about 1–2 nm, and accurate size measurement was not possible. We also could not measure dye distribution in a single DDS nanoparticle by flow cytometry since the nanoparticles were too small. In the absence of suitable luminescence mapping techniques for single particles, we did a microscopic measurement of luminescence intensity of nanoparticle-doped thin film at high optical resolution (using 100X objective); 0.05 mL (0.01 mg/mL) of DDS nanoparticles dispersed in 1 mL 0.1 poly (methylmethacrylate) (PMMA) in toluene was spin coated. Emission intensity was measured at different locations of the film. Our results show that DDS nanoparticles were uniformly dispersed throughout the film, and the average relative intensity was 114.2 with a standard deviation of 2.7 (Figure 4). Using this experimental result, we suggest that DDS nanoparticles are uniform with respect to their luminescence intensity. Each nanoparticle might be treated as a giant dye molecule. 3.1.6.Availability of Pure Silica Surface of DDS NanoparticlesThe availability of the pure silica surface of the DDS nanoparticles has been confirmed by a positive fluorescamine assay.15 The outside silica surface of the DDS nanoparticle has the same properties as that of silica glass, and thus can be easily modified by employing existing silica surface chemistry to attach desired functional groups. To prove this, we first silanized the particle surfaces with TSPDT, a silanization reagent that attaches primary amine groups to the silica surface. A fluorescamine assay, a nonfluorescent molecule that becomes highly fluorescent upon reacting with primary aliphatic amine groups, was used to confirm the amine groups on the surface. Both the pure silica particles prepared by the microemulsion method and the DDS nanoparticles have a similar fluorescence signal enhancement when treated with the same concentration of fluorescamine in dimethylformamide solution (data not shown). 3.2.Application of DDS Nanoparticles as Membrane ProbeThe functionality of the medium size lauroyl-derivatized dye particles as membrane probes has been tested with human leukemia cells. They were then used to stain cell membranes. Optical image (left panel) corresponds well to the fluorescence image (right panel). Figure 5(a) clearly shows that these DDS particles are very effective for membrane staining. Multiple staining experiments showed that the results were reproducible. The control experiment with nonfunctionalized DDS nanoparticles did not show any staining [Figure 5(b)]. Our results demonstrate that lauroyl-derivatized DDS nanoparticles bind well to the cell membrane and can thus be used for cell staining. 4.DiscussionIn this paper we have shown an effective approach for the synthesis of uniform size, spherical dye doped silica nanoparticles. The W/O microemulsion route is an easy and efficient method19 20 21 22 for the preparation of nanoparticles over other existing methodologies.23 24 Typically, microemulsion is a thermodynamically stable homogeneous mixture of three components: oil, water, and surfactant molecules in which the bulk phase is the oil phase. Spherical water nanodroplets remain separated from the bulk oil phase by a thin layer of surfactant molecules and they serve as nanoreactors. The addition of a small amount of NH 4 OH initiates the hydrolysis of TEOS to form silicic acid monomers. These monomers then undergo silica polymerization reaction25 inside the nanoreactor. During the course of polymerization reaction, the dye molecules get doped. Highly luminescent water-soluble dye, Rubpy, thus becomes water insoluble when they are doped inside the silica nanoparticle. Although the water pool size of the microemulsion predominantly determines the size of the resulting particles, the amount of TEOS and the duration of the polymerization reaction also affect their size. The isolation of the DDS nanoparticles from the microemulsion in pure form was also achieved easily by choosing the proper solvent and centrifugation. TEM results (Figure 2) show that the particles are uniform and the dye molecules are doped throughout the silica network. This is due to the fact that the silica polymerization and the nucleation process are much slower in microemulsion than that in aqueous solution. The presence of the heavy atom ruthenium is also seen clearly as black dots (IIb). The redshift in the emission spectra of the DDS nanoparticle is observed when compared to the pure dye in the solid state [Figures 3(b) and 3(c)]. This is due to the presence of silica network surrounding the dye molecules. There is a weak interaction between the silica oxygen atom and the ruthenium ion. This may also explain why there is no dye leaking from the DDS nanoparticles. The self-quenching of the Rubpy dye in the DDS nanoparticles is observed when the amount of dye loading exceeds about 20 wt. Photobleaching experiment and pressure-dependent emission study support that the dye molecules are well protected from the surrounding environment. This also indicates that the silica network is impermeable for oxygen molecule under normal air pressure. The biological membrane consists of phospholipid bilayers. The interior of the membrane is hydrophobic in nature. The polar part of the lipids remains exposed to the intra- and extra-cellular fluids (hydrophilic aqueous cellular medium). Since the membrane is not rigid, small hydrophobic molecules (e.g., pyrene) or any hydrophobic long chain can easily penetrate into it. The driving force of such penetration is the strong hydrophobic–hydrophobic interaction. Based on this knowledge, the DDS nanoparticle membrane probe was designed in such a way that it could bind to the hydrophobic part of the membrane. The modification of the silica surface with the membrane anchoring lauroyl groups was thus appropriate. The demonstration of membrane probing by modified DDS nanoparticles was successful and not limited to this particular application. DDS nanoparticle surface can be modified with a variety of biomolecules (e.g., antibody, DNA, etc.) for specific recognition of biocomponents. 5.ConclusionsLuminescent nanoparticles have been developed using a water-in-oil microemulsion technique. The nanoparticles consist of dye molecules doped throughout the silica network. These dye doped silica nanoparticles can be made in a variety of sizes. They are highly photostable in comparison to most commonly used organic dyes. The silica surface of DDS nanoparticles is available for surface modification and bioconjugation. There are several advantages using DDS nanoparticles for cellular labeling experiments, such as highly photostable dye molecules, small sizes (approximately three orders of magnitude smaller than that of the cell) and noninvasive staining. Further developments, such as direct immunolabeling, multicolored nanoparticles and in situ hybridization, will be important for applications such as cytometry and immunocytobiology. The dye doped nanoparticles can also be versatile for specific and nonspecific labeling and tracing of biocomponents. We are modifying our small size particle’s surface biochemically in such a way that cellular uptake would become possible for the intracellular labeling of a variety of biomolecules. AcknowledgmentsThis work is partially supported by NIH NS39891-01, NSF Career Award, Nanotechnology Initiative BIO-9871880, and Joint Research Fund for Oversea Chinese and Hong Kong Young Scholars, NSFC (20028506). REFERENCES
M. Bruchez Jr.
,
M. Moronne
,
P. Gin
,
S. Weiss
, and
A. P. Alivisatos
,
“Semiconductor nanocrystals as fluorescent biological labels,”
Science , 281 2013
–2016
(1998). Google Scholar
W. C. W. Chan
and
S. M. Nie
,
“Quantum dot bioconjugates for ultrasensitive nonisotropic detection,”
Science , 281 2016
–2018
(1998). Google Scholar
J. R. Taylor
,
M. M. Fang
, and
S. M. Nie
,
“Probing specific sequences on single DNA molecules with bioconjugated fluorescent nanoparticles,”
Anal. Chem. , 72 1979
–1986
(2000). Google Scholar
W. Shenton
,
S. A. Davis
, and
S. Mann
,
“Directed self-assembly of nanoparticles into macroscopic materials using antibody-antigen recognition,”
Adv. Mater. , 11 449
–452
(1999). Google Scholar
R. Elghanian
,
J. J. Storhoff
,
R. C. Mucic
,
R. L. Letsinger
, and
C. A. Mirkin
,
“Selective colorimetric detection of polynucleotides based on the distance-dependent optical properties of gold nanoparticles,”
Science , 277 1078
–1081
(1997). Google Scholar
R. C. Mucic
,
J. J. Storhoff
,
C. A. Mirkin
, and
R. L. Letsinger
,
“DNA-directed synthesis of binary nanoparticle network materials,”
J. Am. Chem. Soc. , 120 12674
–12675
(1998). Google Scholar
G. P. Mitchell
,
C. A. Mirkin
, and
R. L. Letsinger
,
“Programmed assembly of DNA functionalized quantum dots,”
J. Am. Chem. Soc. , 121 8122
–8123
(1999). Google Scholar
H. Harma
,
P. Lehtinen
,
H. Takalo
, and
T. Lovgren
,
“Immunoassay on a single microparticle: The effect of particle size and number on a miniaturized time-resolved fluorometric assay of free prostate-specific antigen,”
Anal. Chim. Acta , 387 11
–19
(1999). Google Scholar
P. Giunchedi
,
U. Conte
,
P. Chetoni
, and
M. F. Saettone
,
“Pectin microspheres as ophthalmic carriers for piroxicam: Evaluation in vitro and in vivo in albino rabbits,”
Eur. J. Pharm. Sci. , 9 1
–7
(1999). Google Scholar
J. Adler
,
A. Jayan
, and
C. D. Melia
,
“A method for quantifying differential expansion within hydrating hydrophilic matrixes by tracking embedded fluorescent microspheres,”
J. Pharm. Sci. , 88 371
–377
(1999). Google Scholar
D. Bourel
,
A. Rolland
,
R. Leverge
, and
B. Genetet
,
“A new immunoreagent for cell labeling-CD3 monoclonal-antibody covalently coupled to fluorescent polymethacrylic nanoparticles,”
J. Immunol. Methods , 106 161
–167
(1988). Google Scholar
J. Cordek
,
X. W. Wang
, and
W. H. Tan
,
“Direct immobilization of glutamate dehydrogenase on optical fiber probes for ultrasensitive glutamate detection,”
Anal. Chem. , 71 1529
–1533
(1999). Google Scholar
X. H. Fang
,
X. J. Liu
,
S. Schuster
, and
W. H. Tan
,
“Designing a novel molecular beacon for surface-immobilized DNA hybridization studies,”
J. Am. Chem. Soc. , 121 2921
–2922
(1999). Google Scholar
X. J. Liu
and
W. H. Tan
,
“A fiberoptic evanescent wave DNA biosensor based on novel molecular beacons,”
Anal. Chem. , 71 5054
–5059
(1999). Google Scholar
L. A. Chung
,
“A fluorescamine assay for membrane protein and peptide samples with non-amino-containing lipids,”
Anal. Biochem. , 248 195
–201
(1997). Google Scholar
P. Hartmann
,
M. J. P. Leiner
, and
M. E. Lippitsch
,
“Response characteristics of luminescent oxygen sensors,”
Sens. Actuators B , 29 251
–257
(1995). Google Scholar
T. Li
,
J. Moon
,
A. A. Morrone
,
J. J. Mecholsky
,
D. R. Talham
, and
J. H. Adair
,
“Preparation of
Ag/SiO2
nanosize composites by a reverse micelle and sol-gel technique,”
Langmuir , 15 4328
–4334
(1999). Google Scholar
S. Shiojiri
,
T. Hirai
, and
I. Komasawa
,
“Immobilization of semiconductor nanoparticles formed in reverse micelles into polyurea via in situ polymerization of diisocyanates,”
Chem. Commun. (Cambridge) , 14 1439
–1440
(1998). Google Scholar
E. Stathatos
,
P. Lianos
,
F. DelMonte
,
D. Levy
, and
D. Tsiourvas
,
“Formation of
TiO2
nanoparticles in reverse micelles and their deposition as thin films on glass substrates,”
Langmuir , 13 4295
–4300
(1997). Google Scholar
S.-Y. Chang
,
L. Liu
, and
S. A. Asher
,
“Preparation and properties of tailored morphology, monodisperse colloidal silica cadmium-sulfide nanocomposites,”
J. Am. Chem. Soc. , 116 6739
–6744
(1994). Google Scholar
“Creation of templated complex topological morphologies in colloidal silica,”
116 6745
–6747
(1994). Google Scholar
M. Li
,
H. Schnablegger
, and
S. Mann
,
“Coupled synthesis and self-assembly of nanoparticles to give structures with controlled organization,”
Nature (London) , 402 393
–395
(1999). Google Scholar
S. S. Davis
,
“Biomedical applications of nanotechnology—implications for drug targeting and gene therapy,”
Trends Biotechnol. , 15 217
–224
(1997). Google Scholar
F. J. Arriagada
and
K. Osseo-Asare
,
“Synthesis of nanosize silica in a nonionic water-in-oil microemulsion: Effect of the water/surfactant molar ratio and ammonia concentration,”
J. Colloid Interface Sci. , 211 210
–220
(1999). Google Scholar
|
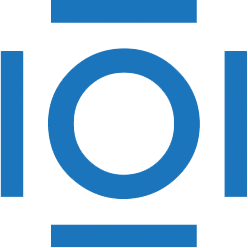
CITATIONS
Cited by 267 scholarly publications and 15 patents.
Nanoparticles
Silica
Luminescence
Molecules
Particles
Polymerization
Transmission electron microscopy